Mind the mitochondria!
Introduction
In critical illness, one of the mainstays of therapeutic interventions is to safeguard adequate oxygen transport to organs and tissues. While in the past the focus mainly was on optimizing macrohemodynamic variables, over the last two decades, the microcirculation has become a prime subject of research and clinical thinking. The dysregulated oxygen transport to tissue in states of shock and resuscitation and the needed interventions to reverse them are still a topic of controversy. Current evidence shows that not a deterioration of systemic variables per se but rather a failure of the microcirculation to transport oxygen to parenchymal cells is the cause of circulatory compromise. Pathological heterogeneity of microcirculatory perfusion leading to functional shunting in the microcirculation causes local hypoxia and reduced cellular respiration, macroscopically manifesting as a reduction in oxygen extraction (1,2).
The defect in oxygen extraction observed during states of sepsis and shock is not only due to alterations in microcirculatory function. In some cases, resuscitation in terms of restored macrohemodynamic and microcirculatory parameters does not lead to clinical improvement and indirect indications of tissue hypoxia, such as high serum lactate, remain. Reduced cellular oxygen consumption, i.e., caused by mitochondrial dysfunction, could mimic such states. Cellular metabolic adaptation could also lead to reduced oxygen extraction and subsequently to a reduced ability of the mitochondria to produce ATP. It is not even unthinkable that under specific circumstances an apparent microcirculatory dysfunction might be an epiphenomenon caused by an in itself adequate adaptation of the microcirculation to an adapted or failing utilization of oxygen and nutrients by parenchymal cells. In any case, mitochondrial alterations in states of shock and sepsis have long been observed (3). If reduced mitochondrial oxygen consumption, either caused by mitochondrial dysfunction due to direct damage or as part of an adaptation mechanism, exists in the presence of normal microcirculatory oxygen transport, then this would have a dramatic impact on the current understanding of resuscitation medicine. Recently, evidence that this might be the case is emerging from both animal and clinical studies. Current resuscitation is entirely focused on promoting oxygen transport to tissues via convection and passive diffusion (4).
Research on the microcirculation in critical illness has been greatly boosted by the emergence of bedside tools that allow direct visualization of the microcirculation of a patient, for example under the tongue (5,6). To further unravel the pathophysiological mechanisms and better understand the interaction between microcirculatory impairment and alterations at the cellular and mitochondrial level we need tools that allow us to look beyond the microcirculation, probing directly aspects of cellular metabolism. Ultimately, the clinician should have access to bedside tools that enable monitoring and verification of treatment success at the microcirculatory, cellular and mitochondrial level. Such tools are currently being developed and tested both in the laboratory and in clinical trials. In this review, we will briefly discuss mitochondrial function, adaptation, causes of dysfunction, the concepts of cytopathic hypoxia and loss of hemodynamic coherence. We will briefly discuss ways to look at the mitochondria in the clinical setting, with a special focus on the novel COMET device. The COMET allows, at the bedside, direct measurement of mitochondrial oxygenation and respiration by an optical technique.
Aspects of mitochondrial function
Mitochondria are double-membrane organelles found in almost all cell types, with the exception of erythrocytes. One of the main functions of mitochondria is to generate adenosine triphosphate (ATP) through oxidative phosphorylation. Over the last two decades, mitochondrial research has undergone a renaissance. Apart from its role in cell bioenergetics, a whole series of discoveries revealed mitochondrial roles in cell death, disease pathology, aging, thermogenesis, oxidative stress, cell signaling and cellular regulation. A review series about the role of mitochondria in aging and various pathophysiological states has been recently published elsewhere (1). The following paragraphs will only provide a short overview of mitochondrial function.
Mitochondria are the primary consumers of oxygen and are responsible for approximately 98% of total body oxygen consumption. Oxygen is ultimately used at complex IV of the electron transport chain in the inner mitochondrial membrane. Reduced nicotinamide adenine dinucleotide (NADH) and flavin adenine dinucleotide (FADH2), generated in the Krebs cycle, are transferred from carrier molecules to the electron transport chain on complex I and II respectively. The resulting electron transport through the chain causes protons to be pumped to the intermembrane space. This proton pumping causes an electrochemical potential over the inner membrane that is used to convert adenosine diphosphate (ADP) to ATP by ATP synthase. ATP is the energy currency of the cells and used for driving cellular processes like maintaining membrane potentials, protein synthesis and replication.
The coupling of mitochondrial respiration to ATP production is not 100% and this leads in part to oxidative phosphorylation generating not only ATP but also reactive oxygen species (ROS). Mitochondria-generated ROS were originally considered potentially toxic by-products, but are now considered to be key signaling molecules in various (patho)physiological processes. For example, mitochondrial ROS at low levels causes metabolic adaptation in hypoxia (2) while high levels of mitochondrial ROS activate apoptosis (3) and autophagy (4). Thus, mitochondrial ROS are associated with processes ranging from cellular adaptation to inducing cell death. Also, mitochondrial ROS are involved in regulating the inflammatory response and can be stimulated by danger signals. An example is the activation of Toll-like receptors (5) by bacterial ligands such as lipopolysaccharide (6).
While most mitochondrial oxygen consumption is used for generating ATP, mitochondrial oxidative metabolism is not limited to ATP generation. As stated above, a small amount of oxygen consumed is directed toward ROS production. Furthermore, a certain amount of respiration is uncoupled from ATP production and lost as heat (7) and used for thermogenesis (8). The amount of mitochondrial coupling varies from tissue to tissue and is typically high in heart and much lower in liver and skeletal muscle (9). Specific uncoupling proteins within the inner mitochondrial membrane use the mitochondrial membrane potential for thermogenesis and are present in e.g., brown adipocytes.
Next to functions linked to the respiratory chain, recent discoveries point to a much broader role of mitochondria in cell homeostasis. For example, mitochondria possess a calcium uniporter (10,11) and might play a role in intracellular calcium homeostasis (12). Also, while mitochondria are essential in sustaining life, they play a major role in (programmed) cell death. Opening of the mitochondrial permeability transition pore, as a result of a stressful stimulus such as calcium or ROS overload, leads to loss of the mitochondrial membrane potential (13). The collapse of the membrane potential results in ATP depletion and necrosis (14), and the release of mitochondrial content such as cytochrome c leads to apoptosis (15).
The role of oxygen
Molecular oxygen is a diatomic gas that constitutes approximately 21% of the volume of air. The electron configuration of the oxygen molecule has two unpaired electrons with the same spin in degenerate orbitals. Therefore, oxygen is paramagnetic and the ground state of oxygen is a triplet state, which is very unusual compared to many other biochemically relevant molecules. The ground state is a triplet state that has the important implication that molecular oxygen does not react directly with many other molecules, in contrast to the highly reactive oxygen radical singlet oxygen.
The adequate supply of oxygen to organs and tissues is of pivotal importance to sustain mammalian life. Aerobic metabolism is maintained through inhalation of air in the lungs and subsequent transport of the absorbed oxygen to tissues via the circulating blood. The flow of hemoglobin-bound oxygen through the macro- and microcirculation and diffusion of molecular oxygen into the tissue cells brings oxygen to its ultimate destination, the mitochondria. In the mitochondria, oxygen is used in oxidative phosphorylation in order to efficiently produce adenosine triphosphate (ATP) that acts as the energy source for many cellular processes. Oxygen also plays a role in many other biochemical processes and mammalian tissue contains a large number of oxygen-consuming enzymes (7), for example for reactive oxygen species generation in signal transduction (8,10).
Cellular hypoxia causes mitochondrial dysfunction, oxidative damage, activation of inflammatory cascades and complement activation, ultimately leading to tissue death. If oxygenation is restored after a prolonged period of time hypoxia, re-oxygenation injury, or ischemia-reperfusion injury, occurs which leads to augmented oxidative stress and tissue injury (11,12).
In this respect it is interesting to note that it is actually unknown, or ill-defined, what “hypoxia” actually is. For example, from a medical point of view one could define hypoxia as a pathological condition in which the body or a region of the body is deprived of an adequate oxygen supply. But the question here obviously is: what does adequate mean in this respect? In the classical view, cellular respiration is unaffected by oxygen levels until PO2 decreases below 2 to 3 mmHg (13-16). This is because of the high affinity of the mitochondrial respiratory chain for oxygen. In the more modern view, oxygen availability is directly influencing cellular metabolism and function by processes like oxygen conformance and cellular metabolic reprogramming, processes that appear to occur at much higher oxygen levels (see below). So, while in the classical view hypoxia is almost equivalent to anoxia, in the modern view any perturbation from a current PO2 level could alter mitochondrial respiration and ultimately cellular function. Therefore, the actions of medical doctors to achieve “adequate tissue oxygenation” might in the end, unintentionally, alter cellular metabolism and function due to alterations in tissue oxygen levels, e.g., by superfluous oxygen administration or vasopressor therapy (17).
Metabolic adaptation
Two important metabolic adaptation mechanisms that may protect a cell from an energy crisis due to reduced oxygen delivery have been described in literature. One is a metabolic shift from aerobic oxidative phosphorylation to an-aerobic glycolysis also referred to as metabolic reprogramming (18). The other is oxygen conformance, which Arthur et al. (19) described as “the ability to reduce energy demand, and hence oxygen consumption, in response to a decline in oxygen availability without a decrease in the concentration of ATP”. Although it is likely that both mechanisms play a role in the adaptation to the reduced supply of oxygen, here the focus will be on oxygen conformance.
Although the mechanism of oxygen conformance was already mentioned by Peter Hochachka in 1986 (20) it was not until 1993 that Schumacker et al. (21) described a decrease in oxygen consumption in primary rat hepatocytes in response to moderate oxygen (20–50 mmHg) deprivation. They showed that the duration of hypoxia and the time period in which oxygen levels were decreased was important for the cellular response, i.e., whether the cells reduced their oxygen consumption rate or not. Before their report, studies had always used a quick depletion of oxygen usually for a short period (several minutes) and shown that the rate of oxygen consumption was oxygen-independent till a PO2 of a few mmHg (22,23). Schumacker et al. (21) confirmed these previous results and showed that in primary rat hepatocytes in which PO2 was rapidly reduced (100 to 0 mmHg in <40 min, oxygen consumption did not change till PO2 was less than 10 mmHg and ATP levels were preserved. In contrast, when PO2 levels were slowly reduced (>2 hr) changes in oxygen consumption already occurred around 70 mmHg with a ~45% decrease in oxygen consumption around 15 mmHg. ATP levels followed the drop in oxygen consumption while NAD(P)H levels increased compared to rapidly deoxygenated cells.
At low O2 tensions of around 20 mmHg the reduced oxygen consumption could be maintained for 18 hours without a decrease in cell viability. The fact that cell viability was preserved during prolonged hypoxia in the slow hypoxia group indicates that the balance between ATP production and consumption was maintained and therefore the cells use less ATP. In a follow-up study Subramanian et al. (24) measured the changes in metabolic demand for ATP during oxygen conformance. They hypothesized whether viability was maintained by preferentially suppressing non-essential processes while leaving essential processes involved in cell homeostasis intact. They confirmed their hypothesis by showing that the non-essential processes of ATP-dependent glucuronidation and sulfation of acetaminophen were decreased significantly during hypoxia. At the same time Na+/K+ pump activity, an essential process for maintaining cell ion homeostasis was maintained. No lactate production was measured during prolonged moderate hypoxia which supports the finding that cellular ATP demand was down-regulated by the reduction of non-essential metabolic processes. Later studies also described oxygen conformance in other cell types such as skeletal muscle (25) and chick cardiomyocytes (26).
The mechanism of oxygen conformance is only partly known. Cytochrome c oxidase (complex 4), the terminal electron acceptor in the electron transport chain, is reversibly inhibited after expose to low oxygen concentration (<50 µM) for several hours (27). It has been suggested that cytochrome C oxidase could act as an oxygen sensor as its reduction state has an effect on its kinetic activity (28) and hence oxygen consumption by the cell. A later study in cardiomyocytes confirmed this mechanism (29). The mechanism involved in the subsequent reduction in ATP as described by Subramanian is less clear. It might involve a difference in ATP affinity of ATP consuming proteins (24). Alternatively AMPK, a kinase that maintains a balance between cellular ATP generation and consumption could be involved (30). It has been shown that AMPK becomes activated during hypoxia and is dependent on mitochondrial complex III (31). It is expected that other causes of ATP reduction, like mitochondrial dysfunction, activate similar pathways to reduce ATP demand.
Hits on mitochondria
Next to their role in cellular adaptation and the more indirect downregulation of mitochondrial respiration, mitochondria are also directly susceptible to certain “hits” that cause mitochondrial damage and dysfunction as part of pathophysiology and treatment. Unfortunately, mitochondrial (dys)function is difficult to measure in the ward and as a consequence there is little clinical awareness of its occurrence. However, general goals in medicine, such as securing oxygen supply, starting therapy for infectious disease, treating cancer, performing an operation and securing comfort during the operation are likely to influence mitochondrial function. For example, acute changes in mitochondrial function might be a cornerstone in the development of SIRS or sepsis. The accompanying mitochondrial dysfunction might be caused by a combination of hyperoxia/hypoxia, medication and inflammation-induced metabolic changes, as shown in Figure 1. A short overview of possible hits on mitochondria follows.
A long standing standard practice on the ward of liberally supplying oxygen to patients led to rise in mortality (32). Setting a more conservative goal for intensive care patients with a saturation between 94% and 98% decreased such negative effect of superfluous oxygen administration (33). Furthermore hyperoxia led to mitochondrial and cellular dysfunction (34). Mitochondria also adapt to hypoxia with a reduction of metabolism (35). Even return of cellular oxygenation after hypoxia induces cellular damage. Reperfusion after cardiac ischemia leads to mitochondrial damage and might be as damaging to cells as the ischemia alone (36).
Medical treatment of diseases or secondary prevention may also lead to mitochondrial side effects. Indeed, in recent years new proof demonstrated the role of mitochondrial damage and/or dysfunction as central players in these side effects. Statins are a typical example of a widely-used drug causing mitochondrial side effects. The basis of preventing cardiovascular disease is lowering of LDL cholesterol, usually successfully achieved by prescribing such statins. However, 5–29% of the patients stop the use of statins due to side effect of statin-induced muscle disease. Current evidence points to mitochondrial dysfunction as an important factor, but the exact process has not been fully understood (37).
Many drugs used in the operating theater and in the ICU are also known for being able to induce mitochondrial alterations, for example propofol. During prolonged sedation a well-known side effect of this drug is the propofol-infusion syndrome; usually characterized by an unexplained high anion gap, metabolic acidosis, rhabdomyolysis and hyperkalemia. Propofol-infusion syndrome is a rare but serious complication, with a recent recommendation to check for mitochondrial disease if this complication develops (38-40). Other drugs commonly encountered in the ICU-setting that potentially cause mitochondrial side effects are antibiotics and chemotherapeutics. Serious side effects, e.g., ototoxicity, are known from bactericidal antibiotics and are linked to mitochondrial dysfunction (41). Chemotherapy-induced cardiotoxicity (42) and myopathy (43) are linked to the oxidative stress on mitochondria caused by these therapies. Although most recognized mitochondria-linked side effects by medication are severe, they are not limited to a single medication group and seem to be widespread. It is unknown if and how medication-induced mitochondrial dysfunction might contribute to the complex pathophysiology seen in ICU patients. Small alterations that in the general population are likely to remain subclinical might add to e.g., sepsis-induced metabolic dysregulation and become an important factor in the progression to multi-organ failure.
In translational research on sepsis, several endotoxemia models in rat and pigs lead to the description of the development of mitochondrial dysfunction over time (44-46). Infectious and inflammatory driven changes in mitochondrial function in humans are not a new phenomenon either (3,47). Because of invasiveness, muscle biopsies, needed for the classical measurement of mitochondrial function in isolated mitochondria, are rarely performed in the ICU. However, differences in development of mitochondrial dysfunction in different muscle types have been shown (48). Also a correlation between mortality, severity of organ dysfunction and mitochondrial dysfunction has been demonstrated in several studies (3,49-51). Although a link has been shown between mitochondrial dysfunction and sepsis, a proof of causality is missing and some argue mitochondrial dysfunction in sepsis might be an epiphenomenon (50). Alternatively, mitochondrial dysfunction and a reduced mitochondrial respiration and ATP production have been suggested to mimic hypoxia, so-called “cytopatic hypoxia” (52).
Cytopathic hypoxia
In intensive care, a primary goal of the intensivist is to preserve organ function or replace it. Corner stones of sepsis treatment on the intensive care are supportive care, safe-guarding a sufficient macrohemodynamic organ perfusion (sufficient mean arterial pressure, lowering of lactate), replacement of organ function (e.g., ventilator support, renal replacement or extracorporal membrane oxygenation), and early antimicrobial therapy. The measurement of microcirculatory function, let alone mitochondrial function, is not part of standard care on the ward and is, as far as we are aware, only performed in research settings. Although lactate is an important factor indicating anaerobic metabolism and therefore tissue hypo-perfusion in sepsis (53), the level of lactate is a crude measure and is known to have pitfalls (54). Cytopathic hypoxia is a concept describing insufficient oxygen metabolism in cells despite sufficient oxygen delivery (55). This apparent tissue hypoxia might need more complex monitoring than lactate and central venous oxygen concentration to effectively monitor its development and reaction to therapy during sepsis (56).
If an infection produces enough inflammatory molecules to produce a SIRS reaction, the first vital signs of a serious infection include tachycardia and hypotension resulting in shock. Macrohemodynamic collapse is prevented by aggressive fluid resuscitation and circulatory support via noradrenaline. However, monitoring the effect of collapse and resuscitation on microcirculatory or mitochondrial function is not part of the standard of care. The return of microcirculation during the resuscitation of a patient with sepsis improves survival (57). In animal models a clear link is also found between a SIRS reaction and mitochondrial dysfunction (44,45,58,59), and early mitochondrial dysfunction in sepsis limits the chance of patient survival (51). Mitochondrial dysfunction in sepsis is not a new concept, as early as in 2002 a direct link between mitochondrial function, organ dysfunction and mortality was described (3).
Aggressive and early fluid resuscitation is one of the pillars of sepsis treatment, with a modest improvement of mortality (60). Mortality is mostly dependent on the amount of organ dysfunction and responsiveness on early treatment goals (60,61).
Preserving vital parameters and microcirculatory perfusion during resuscitation only seems to lead to a modest improvement of mortality. Since mitochondrial dysfunction is linked to an increase in mortality, this results in a complex interaction. On one side, the microcirculation is influenced by the mitochondria of the parenchymal cells and on the other side it is affected by the macrocirculation (4).
The important question then is whether the (apparent) loss of hemodynamic coherence between the macrocirculation and microcirculation should be treated aggressively by hemodynamic measures or that, alternatively, apparent microcirculatory dysfunction should be seen as an epiphenomenon caused by cellular and mitochondrial issues (dysfunction or adaptation). In the latter case, additional attempts to “optimize” macrohemodynamic parameters and microcirculatory perfusion might prove counterproductive. The answer probably varies in different patients and might even change in single patients over the course of the disease and due to given treatments.
Hemodynamic coherence
Resuscitating critically ill patients from different states of shock is key and remains a challenge in critical care. Currently, the main focus is on administration of fluids and vasoactive medication, targeting the normalization of systemic hemodynamic parameters such as blood pressure, cardiac output and venous saturation. However, many randomized trials have failed to show a consistent difference between patient groups that were compared based on interventions directed at these systemic hemodynamic variables (62-66). This could be due to the trial design and trial execution, the intervention, or the heterogeneity of the population. Alternatively, our interventions aimed at improving cellular oxygen delivery might fail to do so or cells were not able to use the delivered oxygen appropriately. Therefore, to further our knowledge about which intervention most benefits the patient, we will need a more physiological, mechanistic and comprehensive approach.
Shock is a condition in which oxygen delivery to the cells and mitochondria is insufficient to sustain cellular activity and thus organ function. We therefore should add targeting microcirculatory and mitochondrial function to our conventional resuscitation targets (i.e., systemic variables). Particularly, we should study whether the condition of shock is already a condition in which systemic and microcirculatory and cellular measures diverge. We also have to study whether our interventions aimed at improving the systemic hemodynamic parameters of a patient are actually improving or maybe worsening our patients microcirculatory and mitochondrial function. Therefore, we should focus on and study this coherence of the macro- and micro and cellular situation with respect to diagnosis and effect of therapeutic interventions.
Hemodynamic coherence is the coherence between the macrocirculation, microcirculation and the parenchymal cells, in which resuscitation procedures aimed at the correction of hemodynamic variables are effective in correction of regional and microcirculatory perfusion and oxygen delivery to the parenchymal cells such that they are able to perform their functional activities in support of organ function (67). There are a number of studies that show that improving systemic macrocirculatory parameters does not lead to improvement of the microcirculation (68,69). This lack of coherence was associated with increased morbidity and mortality (68-70). Additionally, we have to focus on the mitochondrial function in the different states of shock. After all, both the coupling of macrocirculation and microcirculation and the coupling of microcirculation and cellular energetics are impaired in the different states of shock and particularly in sepsis. Van Genderen showed that coherence between macrocirculation and microcirculation existed in an obstructive shock model in pigs, but was lost in a septic shock model (71). Also in septic patients loss of coherence was frequently found (70). In a rat endotoxemia model mitochondrial respiration remained reduced by 38% despite fluid resuscitation restoring mitochondrial oxygenation back to baseline values (45). Such restored oxygenation seems superfluous in comparison to the reduced oxygen demand. Whether this is an example of loss of coherence between cellular oxygen demand and microcirculatory oxygen delivery remains to be clarified.
Furthermore, there are two more dimensions that have to be included in the discussed multidimensional approach. These are the loss of coherence in the different organ systems and the timing of resuscitation and their effect on incoherence and uncoupling. Loss of coherence can vary in the different organ systems. In animal and human studies the intestinal microcirculation particularly remained much longer unresponsive to resuscitation while the macrocirculation and sublingual microcirculation already were restored (72,73). In septic patients the coherence between macrocirculation and different microcirculatory systems could take up to 3 days to occur (73). Controversially, Ospina-Tascon found that the microcirculation was improved by early fluid resuscitation, but was unaffected by resuscitation later in the course (74). Thus, the exact timing of resuscitation and its effect of coherence and coupling of macrocirculation, microcirculation and mitochondrial function over time remain to be elucidated.
Ways to measure mitochondria and current insights
While there is need to assess mitochondrial function in critically ill patients, existing standard technique are invasive and ex vivo (muscle biopsies) and/or cumbersome (NMR), and not readily available 24/7 in a general hospital. Ex vivo techniques that use tissue biopsies have been mainly used to determine mitochondrial dysfunction in patients. From these tissue biopsies, cells or even mitochondria are isolated before actual measurement of oxygen consumption can start. Most common techniques measures oxygen consumption using Clark electrodes (75), like the high resolution respirometer (76). Further evaluation of the mitochondrial respiratory chain is possible by measuring individual complex activity or concentration of the complexes in isolated mitochondria (77).
The location of the muscle biopsy maybe important, since a difference was found between leg muscle and diaphragm (48). Measurement of mitochondrial function is possible in isolated platelets or peripheral blood mononuclear cells (78,79). Of essence in measuring mitochondrial function is the choice of buffer in which the cells are measured, since exposing healthy cells to plasma obtained from patients with sepsis lead to mitochondrial dysfunction (80). In platelets early increase of mitochondrial oxygen metabolism has been linked to an increase in mortality (51,81). Since the location of biopsy and the chosen buffer clearly influence the measured mitochondrial function, interpretation of the results found by respirometers might be difficult. Furthermore, measuring mitochondrial function with ex vivo techniques requires special laboratory equipment (82) and trained staff. Both are not available in our general hospitals, which limits this type of monitoring to a research setting in academic hospitals.
Measurement of aspects of mitochondrial function in vivo is also possible with nuclear magnetic resonance (NMR)-technique and NADH fluorometry (83,84). With the NMR-technique, phosphorus (P) and its transport in the cell can be measured. phosphorus is used by the cell as an energy source (ATP), and thus metabolic flux can be measured. Since a metabolic flux measurement requires use of ATP, an active muscle is required during the NMR measurement.
NADH fluoroscopy measures the NADH fluorescence at 450 nm (blue light). NADH shows fluorescence after photoexcitation with ultraviolet light, but NAD+ does not show such fluorescence. Measuring the ratio between the reduced and oxidized form gives a relative redox potential. While this technique works well in a laboratory setting, e.g., in fluorescence microscopy on single cells, it is very difficult to achieve reliable measurements in vivo.
Both NMR and NADH fluoroscopy measure metabolic state, but the inability to perform bedside monitoring and its costs makes NMR not suitable for standard clinical use in sepsis.
NADH fluoroscopy is available for bedside monitoring and changes in metabolic state are possible in a clinical setting (85). However, standard monitoring of mitochondrial function by NADH fluoroscopy is not yet an option due to sensitivity to artifacts and interpretation issues.
COMET (CellularOxygenMETabolism)
In search for bedside in vivo real-time monitoring of mitochondrial function the Cellular Oxygen METabolism (COMET) monitor was developed (86). The COMET (Photonics Healthcare B.V., Utrecht, The Netherlands) measures mitochondrial oxygen tension (mitoPO2) with delayed fluorescence of mitochondrial protoporphyrin IX (PpIX) (87). Stopping microcirculatory blood flow by pressing the measuring probe on the skin allows the measurement of the mitochondrial oxygen consumption (mitoVO2), here expressed as oxygen disappearance rate (ODR) (88). The ODR measurement is a non-invasive technique to assess mitochondrial respiration in vivo. A first feasibility study with the COMET was performed by our group and mitoPO2 and ODR were measured in healthy volunteers (89). Recently Baumbach et al. showed COMET measurements of mitoPO2, mitoVO2 and mitoDO2 during exercise in healthy volunteers (90). They introduced mitoDO2, a measure for mitochondrial oxygen delivery, as a parameter derived from the dynamics of mitoPO2 during the microvascular reperfusion phase after the release of pressure used for measuring mitoVO2.
MitoPO2 and ODR
The principle of the technique and its development have been described elsewhere (17,87). In short, the COMET monitor uses oxygen-dependent quenching of the delayed fluorescence lifetime of an endogenously synthesized porphyrin, PpIX. Delayed fluorescence of mitochondrial PpIX is a method to measure mitoPO2 in living cells and tissues, non-invasively and feasible in humans. PpIX is the final precursor of heme in the heme biosynthetic pathway. PpIX is synthesized in the mitochondria and administration of 5-aminolevulinc acid (ALA) substantially enhances the PpIX concentration. Photoexcitation of PpIX populates the first excited triplet state, and causes the emission of red delayed fluorescence. The delayed fluorescence lifetime is inversely related to the mitoPO2 according to the Stern–Volmer equation. The background of the delayed fluorescence lifetime technique is extensively described elsewhere (87,91,92).
Clinical example
During a small clinical pilot, part of a larger observational study (IRB approved, CCMO number NL51937.078.15), we measured an example of change in mitochondrial oxygen consumption due to several “hits” on mitochondria. COMET measurements were intraoperatively performed in four patients in the presternal skin region. The patients underwent cytoreductive surgery of peritoneal metastases of a primary intestinal tumor and during the same operation, patients received hyperthermic intraperitoneal chemotherapy (HIPEC). The chemotherapeutic agent was Mitomycin C and was perfused at 42 °C. During surgery and directly post-operative macrohemodynamic support is given by a generous fluid regime and noradrenaline perfusion. An example of the change in ODR during intraperitoneal chemotherapy is shown in Figure 2A. As shown in Figure 2B, in 3 out of 4 patients COMET measured a reduced ODR independently of mitoPO2 after HIPEC perfusion.
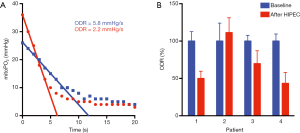
Oxygen balance
Mitochondrial oxygen concentration depends on the concentration of hemoglobin and its saturation, the hemoglobin-oxygen disassociation characteristics in microcirculation and microvascular flow. In essence, the oxygen supply needs to meet the demand to prevent hypoxia and cellular adaptation (93). If oxygen supply exceeds demand it will lead to hyperoxia and oxidative stress induces a cellular response (94). Especially in newborn care, negative effects of hyperoxia on lung development have led to a strategy of permissive hypoxia (95,96). Current clinical practice is safeguarding macrohemodynamics and saturation without knowing the exact cellular oxygen concentration (97). Mitochondria are the oxygen consumers, thus maintaining a sufficient oxygen concentration in the mitochondria is the goal in the blood-tissue oxygen distribution. Therefore, mitoPO2 is the result of the balance between oxygen supply and the cells ability to consume it (as shown in Figure 3).
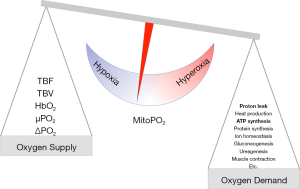
Conclusions
Since a primary goal of caregivers is maintaining supply and demand, mitochondrial oxygen levels and oxygen consumption might be useful parameters for monitoring the effectiveness of therapies in optimizing oxygen supply. Achieving oxygen hemostasis, and thus maintaining cellular oxygen concentration and oxygen metabolism within a normal range may be an ultimate goal. COMET is able to provide insight in the oxygen balance at tissue level, and thus provides important information about aspects of mitochondrial function. Considering the complexity of the pathophysiology of a critically ill patient, especially the patients with severe sepsis and septic shock, a multilevel approach is needed. In understanding the interplay between macrocirculation, microcirculation and parenchymal cells the mitochondria are key players that should not be overlooked.
Acknowledgments
None.
Footnote
Conflicts of Interest: EG Mik is inventor of the technique for measuring mitochondrial oxygen tension, as used in the COMET measuring system. He is founder and shareholder of Photonics Healthcare B.V., Utrecht, The Netherlands. Photonics Healthcare develops and markets the COMET system. Other authors declare no conflict of interest.
Ethical Statement: The authors are accountable for all aspects of the work in ensuring that questions related to the accuracy or integrity of any part of the work are appropriately investigated and resolved.
References
- Ince C, Ashruf JF, Avontuur JA, et al. Heterogeneity of the hypoxic state in rat heart is determined at capillary level. Am J Physiol 1993;264:H294-301. [PubMed]
- Ince C, Sinaasappel M. Microcirculatory oxygenation and shunting in sepsis and shock. Crit Care Med 1999;27:1369-77. [Crossref] [PubMed]
- Brealey D, Brand M, Hargreaves I, et al. Association between mitochondrial dysfunction and severity and outcome of septic shock. Lancet 2002;360:219-23. [Crossref] [PubMed]
- Ince C, Mik EG. Microcirculatory and mitochondrial hypoxia in sepsis, shock, and resuscitation. J Appl Physiol 2016;120:226-35. [Crossref] [PubMed]
- Donati A, Damiani E, Domizi R, et al. Alteration of the sublingual microvascular glycocalyx in critically ill patients. Microvasc Res 2013;90:86-9. [Crossref] [PubMed]
- Klijn E, Uil Den CA, Bakker J, et al. The Heterogeneity of the Microcirculation in Critical Illness. Clin Chest Med 2008;29:643-54. [Crossref] [PubMed]
- Vanderkooi JM, Erecińska M, Silver IA. Oxygen in mammalian tissue: methods of measurement and affinities of various reactions. Am J Physiol 1991;260:C1131-50. [Crossref] [PubMed]
- Dröge W. Free radicals in the physiological control of cell function. Physiol Rev 2002;82:47-95. [Crossref] [PubMed]
- Rolfe DF, Brown GC. Cellular energy utilization and molecular origin of standard metabolic rate in mammals. Physiol Rev 1997;77:731-58. [Crossref] [PubMed]
- Xi Q, Cheranov SY, Jaggar JH. Mitochondria-derived reactive oxygen species dilate cerebral arteries by activating Ca2+ sparks. Circ Res 2005;97:354-62. [Crossref] [PubMed]
- Panisello-Roselló A, Roselló-Catafau J. Molecular Mechanisms and Pathophysiology of Ischemia-Reperfusion Injury. Int J Mol Sci 2018;19:4093. [Crossref] [PubMed]
- Tan S, Yokoyama Y, Wang Z, et al. Hypoxia-reoxygenation is as damaging as ischemia-reperfusion in the rat liver. Crit Care Med 1998;26:1089-95. [Crossref] [PubMed]
- Jones DP, Mason HS. Gradients of O2 concentration in hepatocytes. J Biol Chem 1978;253:4874-80. [PubMed]
- Longmuir IS. Respiration rate of rat-liver cells at low oxygen concentrations. Biochem J 1957;65:378-82. [Crossref] [PubMed]
- Wilson DF, Rumsey WL, Green TJ, et al. The oxygen dependence of mitochondrial oxidative phosphorylation measured by a new optical method for measuring oxygen concentration. J Biol Chem 1988;263:2712-8. [PubMed]
- Wilson DF, Erecińska M, Drown C, et al. The oxygen dependence of cellular energy metabolism. Arch Biochem Biophys 1979;195:485-93. [Crossref] [PubMed]
- Mik EG. Measuring Mitochondrial Oxygen Tension: From Basic Principles to Application in Humans. Anesth Analg 2013;117:834-46. [Crossref] [PubMed]
- Schito L, Rey S. Cell-Autonomous Metabolic Reprogramming in Hypoxia. Trends Cell Biol 2018;28:128-42. [Crossref] [PubMed]
- Arthur PG, Giles JJ, Wakeford CM. Protein synthesis during oxygen conformance and severe hypoxia in the mouse muscle cell line C2C12. Biochim Biophys Acta. 2000;1475:83-9. [Crossref] [PubMed]
- Hochachka PW. Defense strategies against hypoxia and hypothermia. Science 1986;231:234-41. [Crossref] [PubMed]
- Schumacker PT, Chandel N, Agusti AG. Oxygen conformance of cellular respiration in hepatocytes. Am J Physiol 1993;265:L395-402. [PubMed]
- Jones DP, Kennedy FG. Intracellular oxygen supply during hypoxia. Am J Physiol 1982;243:C247-53. [Crossref] [PubMed]
- Wilson DF, Erecińska M, Nuutinen EM, et al. Dependence of cellular metabolism and local oxygen delivery on oxygen tension. Adv Exp Med Biol 1984;180:629-34. [Crossref] [PubMed]
- Subramanian RM, Chandel N, Budinger GRS, et al. Hypoxic conformance of metabolism in primary rat hepatocytes: a model of hepatic hibernation. Hepatology 2007;45:455-64. [Crossref] [PubMed]
- Hogan MC, Kurdak SS, Arthur PG. Effect of gradual reduction in O2 delivery on intracellular homeostasis in contracting skeletal muscle. J Appl Physiol 1996;80:1313-21. [Crossref] [PubMed]
- Budinger GR, Chandel N, Shao ZH, et al. Cellular energy utilization and supply during hypoxia in embryonic cardiac myocytes. Am J Physiol 1996;270:L44-53. [PubMed]
- Chandel NS, Budinger GR, Schumacker PT. Molecular oxygen modulates cytochrome c oxidase function. J Biol Chem 1996;271:18672-7. [Crossref] [PubMed]
- Chandel NS, Budinger GR, Choe SH, et al. Cellular respiration during hypoxia. Role of cytochrome oxidase as the oxygen sensor in hepatocytes. J Biol Chem 1997;272:18808-16. [Crossref] [PubMed]
- Budinger GR, Duranteau J, Chandel NS, et al. Hibernation during hypoxia in cardiomyocytes. Role of mitochondria as the O2 sensor. J Biol Chem. 1998;273:3320-6. [Crossref] [PubMed]
- Hardie DG. AMP-activated/SNF1 protein kinases: conserved guardians of cellular energy. Nat Rev Mol Cell Biol 2007;8:774-85. [Crossref] [PubMed]
- Emerling BM, Weinberg F, Snyder C, et al. Hypoxic activation of AMPK is dependent on mitochondrial ROS but independent of an increase in AMP/ATP ratio. Free Radic Biol Med 2009;46:1386-91. [Crossref] [PubMed]
- Damiani E, Adriario E, Girardis M, et al. Arterial hyperoxia and mortality in critically ill patients: a systematic review and meta-analysis. Crit Care 2014;18:711. [Crossref] [PubMed]
- Girardis M, Busani S, Damiani E, et al. Effect of Conservative vs Conventional Oxygen Therapy on Mortality Among Patients in an Intensive Care Unit. JAMA 2016;316:1583-9. [Crossref] [PubMed]
- Resseguie EA, Staversky RJ, Brookes PS, et al. Hyperoxia activates ATM independent from mitochondrial ROS and dysfunction. Redox Biol 2015;5:176-85. [Crossref] [PubMed]
- Fuhrmann DC, Brüne B. Mitochondrial composition and function under the control of hypoxia. Redox Biol 2017;12:208-15. [Crossref] [PubMed]
- Hüttemann M, Helling S, Sanderson TH, et al. Regulation of mitochondrial respiration and apoptosis through cell signaling: cytochrome c oxidase and cytochrome c in ischemia/reperfusion injury and inflammation. Biochim Biophys Acta 2012;1817:598-609. [Crossref] [PubMed]
- Ramachandran A, Patra S, Balasubramanian KA. Intestinal Mitochondrial Dysfunction in Surgical Stress. J Surg Res 2001;99:120-8. [Crossref] [PubMed]
- Finsterer J, Frank M. Propofol Is Mitochondrion-Toxic and May Unmask a Mitochondrial Disorder. J Child Neurol 2016;31:1489-94. [Crossref] [PubMed]
- Hemphill S, McMenamin L, Bellamy MC, et al. Propofol infusion syndrome: a structured literature review and analysis of published case reports. Br J Anaesth 2019;122:448-59. [Crossref] [PubMed]
- Vollmer JP, Haen S, Wolburg H, et al. Propofol Related Infusion Syndrome: Ultrastructural Evidence for a Mitochondrial Disorder. Crit Care Med 2018;46:e91-4. [Crossref] [PubMed]
- Kalghatgi S, Spina CS, Costello JC, et al. Bactericidal antibiotics induce mitochondrial dysfunction and oxidative damage in Mammalian cells. Sci Transl Med 2013;5:192ra85. [Crossref] [PubMed]
- Gorini S, De Angelis A, Berrino L, et al. Chemotherapeutic Drugs and Mitochondrial Dysfunction: Focus on Doxorubicin, Trastuzumab, and Sunitinib. Oxid Med Cell Longev 2018;2018:7582730. [Crossref] [PubMed]
- Guigni BA, Callahan DM, Tourville TW, et al. Skeletal muscle atrophy and dysfunction in breast cancer patients: role for chemotherapy-derived oxidant stress. Am J Physiol Cell Physiol 2018;315:C744-56. [Crossref] [PubMed]
- Protti A, Carré J, Frost MT, et al. Succinate recovers mitochondrial oxygen consumption in septic rat skeletal muscle. Crit Care Med 2007;35:2150-5. [Crossref] [PubMed]
- Harms FA, Bodmer SIA, Raat NJH, et al. Non-invasive monitoring of mitochondrial oxygenation and respiration in critical illness using a novel technique. Crit Care 2015;19:343. [Crossref] [PubMed]
- Sun H, Huang Y, Yin C, et al. Lipopolysaccharide markedly changes glucose metabolism and mitochondrial function in the longissimus muscle of pigs. Animal 2016;10:1204-12. [Crossref] [PubMed]
- Levy RJ. Mitochondrial dysfunction, bioenergetic impairment, and metabolic down-regulation in sepsis. Shock 2007;28:24-8. [Crossref] [PubMed]
- Fredriksson K, Rooyackers O. Mitochondrial function in sepsis: respiratory versus leg muscle. Crit Care Med 2007;35:S449-53. [Crossref] [PubMed]
- Callahan LA, Supinski GS. Sepsis Induces Diaphragm Electron Transport Chain Dysfunction and Protein Depletion. Am J Respir Crit Care Med 2005;172:861-8. [Crossref] [PubMed]
- Fink MP. Cytopathic hypoxia and sepsis: is mitochondrial dysfunction pathophysiologically important or just an epiphenomenon. Pediatr Crit Care Med 2015;16:89-91. [Crossref] [PubMed]
- Puskarich MA, Kline JA, Watts JA, et al. Early alterations in platelet mitochondrial function are associated with survival and organ failure in patients with septic shock. J Crit Care 2016;31:63-7. [Crossref] [PubMed]
- Fink MP. Cytopathic hypoxia. Is oxygen use impaired in sepsis as a result of an acquired intrinsic derangement in cellular respiration? Crit Care Clin 2002;18:165-75. [Crossref] [PubMed]
- Jansen TC, van Bommel J, Schoonderbeek FJ, et al. Early Lactate-Guided Therapy in Intensive Care Unit Patients. Am J Respir Crit Care Med 2010;182:752-61. [Crossref] [PubMed]
- Hernandez G, Bellomo R, Bakker J. The ten pitfalls of lactate clearance in sepsis. Intensive Care Med 2019;45:82-5. [Crossref] [PubMed]
- Fink M. Cytopathic hypoxia in sepsis. Acta Anaesthesiol Scand Suppl 1997;110:87-95. [Crossref] [PubMed]
- Fink MP. Bench-to-bedside review: Cytopathic hypoxia. Crit Care 2002;6:491-9. [Crossref] [PubMed]
- Charlton M, Sims M, Coats T, et al. The microcirculation and its measurement in sepsis. J Intensive Care Soc 2017;18:221-7. [Crossref] [PubMed]
- Herminghaus A, Barthel F, Heinen A, et al. Severity of polymicrobial sepsis modulates mitochondrial function in rat liver. Mitochondrion 2015;24:122-8. [Crossref] [PubMed]
- Herminghaus A, Papenbrock H, Eberhardt R, et al. Time-related changes in hepatic and colonic mitochondrial oxygen consumption after abdominal infection in rats. Intensive Care Med Exp 2019;7:4. [Crossref] [PubMed]
- Rhodes A, Phillips G, Beale R, et al. The Surviving Sepsis Campaign bundles and outcome: results from the International Multicentre Prevalence Study on Sepsis (the IMPreSS study). Intensive Care Med 2015;41:1620-8. [Crossref] [PubMed]
- Jessen MK, Skibsted S, Shapiro NI. Number of organ dysfunctions predicts mortality in emergency department patients with suspected infection. Eur J Emerg Med 2017;24:176-82. [Crossref] [PubMed]
- Mouncey PR, Osborn TM, Power GS, et al. Trial of early, goal-directed resuscitation for septic shock. N Engl J Med 2015;372:1301-11. [Crossref] [PubMed]
- ProCESS Investigators, Yealy DM, Kellum JA, et al. A randomized trial of protocol-based care for early septic shock. N Engl J Med 2014;370:1683-93. [Crossref] [PubMed]
- ARISE Investigators, ANZICS Clinical Trials Group, Peake SL, et al. Goal-directed resuscitation for patients with early septic shock. N Engl J Med 2014;371:1496-506. [Crossref] [PubMed]
- Holst LB, Haase N, Wetterslev J, et al. Lower versus higher hemoglobin threshold for transfusion in septic shock. N Engl J Med 2014;371:1381-91. [Crossref] [PubMed]
- Asfar P, Meziani F, Hamel J-F, et al. High versus low blood-pressure target in patients with septic shock. N Engl J Med 2014;370:1583-93. [Crossref] [PubMed]
- Ince C. Hemodynamic coherence and the rationale for monitoring the microcirculation. Crit Care 2015;19 Suppl 3:S8. [PubMed]
- De Backer D, Donadello K, Sakr Y, et al. Microcirculatory alterations in patients with severe sepsis: impact of time of assessment and relationship with outcome. Crit Care Med 2013;41:791-9. [Crossref] [PubMed]
- Trzeciak S, McCoy JV, Phillip Dellinger R, et al. Early increases in microcirculatory perfusion during protocol-directed resuscitation are associated with reduced multi-organ failure at 24 h in patients with sepsis. Intensive Care Med 2008;34:2210-7. [Crossref] [PubMed]
- Sakr Y, Dubois M-J, De Backer D, et al. Persistent microcirculatory alterations are associated with organ failure and death in patients with septic shock. Crit Care Med 2004;32:1825-31. [Crossref] [PubMed]
- van Genderen ME, Klijn E, Lima A, et al. Microvascular perfusion as a target for fluid resuscitation in experimental circulatory shock. Crit Care Med 2014;42:e96-105. [Crossref] [PubMed]
- Edul VSK, Ince C, Navarro N, et al. Dissociation between sublingual and gut microcirculation in the response to a fluid challenge in postoperative patients with abdominal sepsis. Ann Intensive Care 2014;4:39. [Crossref] [PubMed]
- Boerma EC, van der Voort PHJ, Spronk PE, et al. Relationship between sublingual and intestinal microcirculatory perfusion in patients with abdominal sepsis. Crit Care Med 2007;35:1055-60. [Crossref] [PubMed]
- Ospina-Tascon G, Neves AP, Occhipinti G, et al. Effects of fluids on microvascular perfusion in patients with severe sepsis. Intensive Care Med 2010;36:949-55. [Crossref] [PubMed]
- Estabrook RW. Mitochondrial respiratory control and the polarographic measurement of ADP:O ratios. Methods Enzymol 1967;10:41-7. [Crossref]
- Gnaiger E, Steinlechner-Maran R, Méndez G, et al. Control of mitochondrial and cellular respiration by oxygen. J Bioenerg Biomembr 1995;27:583-96. [Crossref] [PubMed]
- Scholte HR, Busch HF, Bakker HD, et al. Riboflavin-responsive complex I deficiency. Biochim Biophys Acta 1995;1271:75-83. [Crossref] [PubMed]
- Sjövall F, Ehinger JKH, Marelsson SE, et al. Mitochondrial respiration in human viable platelets—Methodology and influence of gender, age and storage. Mitochondrion 2013;13:7-14. [Crossref] [PubMed]
- Jang DH, Orloski CJ, Owiredu S, et al. Alterations in Mitochondrial Function in Blood Cells Obtained from Patients with Sepsis Presenting to an Emergency Department. Shock 2019;51:580-4. [Crossref] [PubMed]
- Trentadue R, Raffaella T, Fiore F, et al. Induction of mitochondrial dysfunction and oxidative stress in human fibroblast cultures exposed to serum from septic patients. Life Sci 2012;91:237-43. [Crossref] [PubMed]
- Sjövall F, Morota S, Hansson MJ, et al. Temporal increase of platelet mitochondrial respiration is negatively associated with clinical outcome in patients with sepsis. Crit Care 2010;14:R214. [Crossref] [PubMed]
- Sperl W, Skladal D, Gnaiger E, et al. High resolution respirometry of permeabilized skeletal muscle fibers in the diagnosis of neuromuscular disorders. Mol Cell Biochem 1997;174:71-8. [Crossref] [PubMed]
- Mayevsky A, Barbiro-Michaely E. Use of NADH fluorescence to determine mitochondrial function in vivo. Int J Biochem Cell Biol 2009;41:1977-88. [Crossref] [PubMed]
- Alves TC, Jarak I, Carvalho RA. NMR Methodologies for Studying Mitochondrial Bioenergetics. Methods Mol Biol 2012;810:281-309. [Crossref] [PubMed]
- Mayevsky A, Chance B. Oxidation-reduction states of NADH in vivo: from animals to clinical use. Mitochondrion 2007;7:330-9. [Crossref] [PubMed]
- Ubbink R, Bettink MAW, Janse R, et al. A monitor for Cellular Oxygen METabolism (COMET): monitoring tissue oxygenation at the mitochondrial level. J Clin Monit Comput 2017;31:1143-50. [Crossref] [PubMed]
- Mik EG, Stap J, Sinaasappel M, et al. Mitochondrial PO2 measured by delayed fluorescence of endogenous protoporphyrin IX. Nat Methods 2006;3:939-45. [Crossref] [PubMed]
- Harms FA, Bodmer SIA, Raat NJH, et al. Cutaneous mitochondrial respirometry: non-invasive monitoring of mitochondrial function. J Clin Monit Comput 2015;29:509-19. [Crossref] [PubMed]
- Harms FA, Stolker RJ, Mik EG. Cutaneous Respirometry as Novel Technique to Monitor Mitochondrial Function: A Feasibility Study in Healthy Volunteers. PLoS One 2016;11:e0159544. [Crossref] [PubMed]
- Baumbach P, Neu C, Derlien S, et al. A pilot study of exercise-induced changes in mitochondrial oxygen metabolism measured by a cellular oxygen metabolism monitor (PICOMET). Biochim Biophys Acta Mol Basis Dis 2019;1865:749-58. [Crossref] [PubMed]
- Mik EG, Johannes T, Zuurbier CJ, et al. In Vivo Mitochondrial Oxygen Tension Measured by a Delayed Fluorescence Lifetime Technique. Biophys J 2008;95:3977-90. [Crossref] [PubMed]
- Harms FA, Bodmer SIA, Raat NJH, et al. Validation of the protoporphyrin IX-triplet state lifetime technique for mitochondrial oxygen measurements in the skin. Opt Lett 2012;37:2625-7. [Crossref] [PubMed]
- Solaini G, Baracca A, Lenaz G, et al. Hypoxia and mitochondrial oxidative metabolism. Biochim Biophys Acta 2010;1797:1171-7. [Crossref] [PubMed]
- Lee PJ, Choi AMK. Pathways of cell signaling in hyperoxia. Free Radic Biol Med 2003;35:341-50. [Crossref] [PubMed]
- Carlo WA. Permissive hypercapnia and permissive hypoxemia in neonates. J Perinatol 2007;27:S64-70. [Crossref]
- Maltepe E, Saugstad OD. Oxygen in health and disease: regulation of oxygen homeostasis--clinical implications. Pediatr Res 2009;65:261-8. [Crossref] [PubMed]
- Mik EG. Hyperbaric oxygen preconditioning: what remains between hypoxia and hyperoxia? Clin Exp Pharmacol Physiol 2011;38:656-7. [Crossref] [PubMed]
Cite this article as: Wefers Bettink MA, Arbous MS, Raat NJ, Mik EG. Mind the mitochondria! J Emerg Crit Care Med 2019;3:45.