Pathophysiology of ischemia-reperfusion injury and its management with hyperbaric oxygen (HBO): a review
Introduction
Acute trauma is one of the leading causes of morbidity and mortality in the world. Its global burden of disease increases by the year. Trauma may involve all the layers of tissue and become a very complex injury. Direct mechanical insult to tissues may result in physiological and immunologic disturbances. An inappropriate response leads to a cytokine, immunological and genomic storms that contribute to the deleterious effects of acute trauma. If not resolve promptly and adequately, the lesion will become an ischemia-reperfusion injury (IRI) and eventually lead to tissue loss and/or death (1).
It appears that IRI is the common tissue response to acute insults and relates to its morbidity and mortality. It has been described to participate in several conditions like ischemic stroke, acute myocardium infarction, cardiac arrest, burns, and trauma. The acute interruption of blood and/or oxygen supply creates an imbalance between demand and supply. After sometime, the oxygen re-entry upon reperfusion, may lead to a burst of oxidative damage mediated by the production of reactive oxygen species (ROS) and reduction of antioxidant reserves of the cells, tissues, organs or body. Oxidative stress starts in the hypoxic stage but is augmented in the reperfusion injury. If sustained for a period of hours, oxidative stress will generate an oxidative damage that may lead into multiple organ failure (MOF) and eventually death. IRI could be also named as hypoxic re-oxygenation injury. It is referred as the oxygen paradox in which reoxygenation of an ischemic tissue produces injury that greatly exceeds the one created by ischemia alone.
Several methods have been used to reduce IRI. Between those reported are: antioxidants to reduce oxidative stress, free radical scavengers to remove metabolic waste, and hypoxic preconditioning. All have some beneficial effects but have lacked to prove effectiveness when applied during the reperfusion stage (2). When hyperbaric oxygen therapy (HBOT) is used within the window of opportunity (<6 h), it modifies local and systemic hypoxia, preserves the marginal tissue, reduces the inflammatory cascades and helps to reduce the ischemic and metabolic penumbras; enhancing the survival of the affected tissue or organ (3).
Pathophysiology of IRI
In acute lesions the core of the pathophysiology in the first 72 h is the ischemia (hypoxia)/reperfusion (re-oxygenation) (IR) injury. It is characterized by the local consumption of oxygen and nutrients that generate and ischemic and metabolic penumbra. These penumbras could be reversible or irreversible depending on the degree and duration of the hypoxia. Time is a key factor, the longer it takes to restore the flow and oxygen tension; the larger the tissue lost. So, time is tissue. The reversibility of the IRI depends on the mitochondrial ability to maintain ATP production (4).
Once the production of ATP is stopped, there is a dysfunction of the membrane ion pumps (Na-K and K-Ca), originating cytotoxic edema. When the mitochondrial dysfunction is severe, the mitochondrial transition pore opens and calcium is released into the cytoplasm and becomes the first inflammatory mediator. Also, cytochrome C is released initiating the intrinsic apoptotic cascade (5,6).
The central part (20%) of the lesion will be lost to necrosis. The surrounding area suffers ischemia, hypoxia, and edema. This is the area of the recoverable marginal tissue. It is also known as the area of penumbra. It constitutes close to 80% of the damaged tissue in IRI. If the ischemic and metabolic penumbras are not promptly and adequately recovered; the damage will extend and deepen due to IRI and apoptosis (3).
Mitochondria in IRI
Lack of blood supply deprives the cells of necessary glucose and oxygen, and disturbs cellular homeostasis, culminating in cell death. It is important to restore early the ischemic penumbra. Tissue plasminogen (tPA) is the only effective pharmacological therapy approved by the Food and Drug Administration (FDA) for acute ischemic stroke since 1996, but its use remains limited due to the narrow therapeutic window. Accumulating evidences indicate that the maintaining the mitochondria is crucial for cell survival and tissue improvement (7).
In acute pathologies, mitochondria produce oxidative stress and damage that contributes to retrograde redox signaling from the organelle to the cytosol and nucleus. During hypoxia there are two modes of operation of isolated mitochondria that result in the significant superoxide production from complex I. One, when there is no production of adenosine tri-phosphate (ATP) and consequently have a high promoting force (Δp) and a reduce coenzyme Q (CoQ) pool; and two, when there is a high NADH/NAD+ ratio in the mitochondria matrix (8).
During cellular ischemia and/or hypoxia, the cell undergoes seven stages of shock. The first four are reversible. The reversibility depends on cells’ capability to maintain ATP production by the mitochondria (4). Mitochondrial production is reported to increase in very low oxygen (O2) conditions; when O2 is decreased from 21% to 1–3%. Once the ATP production reaches a critical level (<1 mol/kg), the cell enters an energy crisis (9). It appears that during hypoxia, the increased mitochondrial ROS production is mediated principally by hypoxia-induced factor-1α (HIF-1α) (8).
Once the cells enter an energy crisis during hypoxia, there is an interruption of passage of protons through the mitochondrial complexes and at the ATPase-synthase. The protons migrate to the center of the cristae and produce increase of ROS production and oxidative stress. It is in this stage that an increase of neural nitric oxide synthase (nNOS) and inducible nitric oxide synthase (iNOS) production happen (8). If this oxidative stress is maintained for certain time it will lead to oxidative damage. This damage promotes the intrinsic apoptotic pathways with the production of caspases. Again, it is a reversible process until there is production of caspase 3 and the liberation of cytochrome C, when it becomes irreversible. During the energy crisis there is also an increase production of glutamate, the central nervous systems (CNS) excitatory transmitter (3,10).
Mitochondria are the only cell organelle that has deoxyribonucleic acid (DNA) and it shares around 30% of it with the nucleus. Mitochondria are not only important for ATP production; it generates in the intrinsic apoptosis pathway, chelates Ca2+ from the cytosol, and participates in quick responses during acute stress in conjunction with the nucleus. It is also probable that ROS production by mitochondria is important in maintaining the redox state of the cell and promote cell-to-cell communication. Redox signaling can occur by mitochondria releasing hydrogen peroxide (H2O2) that modulates the activity of target proteins through the reversible oxidation of critical protein thiols, thus altering the activity of enzymes, kinases, phosphatases and transcription factors in mitochondria, cytosol and nucleus (8).
With mitochondrial dysfunction and depletion of ATP, there is a loss of Na+/K+ and Ca2+ membrane pumps, which creates ionic misbalance and cytotoxic edema and membrane depolarization. The release of cytochrome C occurs immediately before total mitochondrial dysfunction happens, probably due to the rise of nitric oxide. The opening of the mitochondrial transition pore follows. Once the pore is open, it releases the mitochondria calcium content into the cytosol; increasing cellular edema and affecting cellular homeostasis and inflammation, leading to cellular death (3,7,11,12).
Inflammation in IRI
Once calcium is liberated outside the cell, it becomes the first modulator of inflammatory cascades (13). One of the cascades is mediated by the conversion of xanthine dehydrogenase to xanthine oxidase, which promotes the production of ROS (14). Another is mediated by the arachidonic acid with the subsequent elevation of cyclooxygenase (COX), lipoxygenase, leukotriene, thromboxane and prostaglandins (15). Another cascade is mediated by nuclear transition factor kappa B (NF-κB). It enhances the production of endothelins and cytokines (IL-1, IL-6, IL-8, TNF-α) (15-17). Other generates the elevation of interferon-γ, glutamate, caspases (3,8,9), HIF-1α, and nitric oxide derived from iNOS (16).
Endothelium is a large and important organ that participates in inflammation (18). It integrates many functions and serves as an alarm system. Ischemia, hypoxia and hypoglycemia increase the expression of adhesion molecules of the endothelium [intercellular adhesion molecule (ICAM), vascular cell adhesion molecule (VCAM)] and of the neutrophils (integrin β2) (19,20). In the latter phase (reperfusion) of the IRI, the expression of endothelial and neutrophil adhesion molecules are responsible for the migration of neutrophils into the wound, where they liberate ROS and promote inflammation. ROS may produce an arteriolar vasoconstriction, promoting a no-flow state (IRI) observed in the first 72 h in re-implantation of limbs and skin grafts or flaps (18-20). Thus, it appears that maintaining adequate perfusion and the cellular metabolic needs may be the cornerstone to reduce damage and promote early cell protection (3,21).
In severe cases, the excessive inflammatory response observed in systemic IRI generates a massive production of inflammatory mediators (cytokines storm); that is followed by an over expression of inflammatory genes (genomic storm). At this stage, IRI is potentiated by the immune system. It appears that toll like receptors (TLRs) participate in several inflammation pathways. TLRs are transmembrane proteins that have a central role in recognizing endogenous ligands, such as those released from IR damaged tissue. The family of human TLR consists of 10 members. All possess a leucine-rich repeat sequence in the extracellular domain; and a Toll/interleukin (IL-1) receptor in the intracellular domain. When a cell is damaged it liberates endogenous particles that bind to TLR2, TLR4 and TLR9. The end result is further damage by subsequent inflammatory reactions and activation of the innate immune system. The effectors in IRI are cytokines, chemokines, micro-ribonucleic acid (miRNA) and others mediators that exacerbates tissue inflammation and injury through neutrophilic and T-lymphocytic involvement (22-25).
The complement system in IRI
The first complement mediated injury studies were performed in a myocardial ischemia model. C3 cleavage was related to chemotaxis and leukocyte activation. Presently, it is known that during IRI, complement activation happens in several organs (heart, skeletal muscles, kidneys, lungs, etc.) (18). In skeletal muscle IRI models showed there was a reduction of deposition of complement C3 suggesting a participation of antibody mediated classical pathway activation. It has been reported there is C3b deposition (classical pathway) in stressed human endothelial cells in the absence of immune complexes. It also appears that complement could be activated by a C2-dependent mechanism (alternative pathway activation). In low oxygen tension (hypoxia), there was a need for C2 in the activation of complement on human endothelial cells in vitro; and for the deposition of C3b. It seems that there is a role of classical, lectin and alternative pathways in IRI (18,26).
In the IRI injuries in the CNS (stroke) the only early-accepted treatments used to modify the ischemic penumbra are stenting, angioplasty, hypothermia, and thrombolysis, especially when applied in the first 3 to 5 h of the onset of IRI (window of opportunity). The FDA has only accepted the plasminogen activator [recombinant tissue plasminogen activator (rtPA)]. None of these have proven to resolve the metabolic penumbra. Medication has not shown protective effects either (27).
Presently, it is suggested that there are several epigenetic processes that could participate in the pathophysiology of IRI. Vascular or neurological regeneration may be mediated by DNA methylation, histone deacetylase, histone methylation and miRNAs. They have become important biomarkers for IRI, stroke and other related pathologies. Epigenetic strategies for IRI treatment might modulate cell regeneration, promote repair, and functional recovery (28).
Hyperbaric oxygen (HBO) in IRI
HBOT is the treatment where the patient (whole body) is put inside a treatment chamber at a pressure higher than 1.4 atmospheres absolute (atm abs); and breathes 100% oxygen. The treatment chamber could be monoplace (one patient) or multiplace (more than one patient) (3,29).
Although HBOT is based in all the gas laws, the most important is Henry’s law. It addresses gas solubility in a fluid. The amount of gas dissolved will increase depending on the partial pressure of the gas breathed. The higher the pressure used, the higher the solubility obtained. The normal range of pressures employed is between 1.5 and 3.0 atm abs. The normal treatment duration would be between 45 and 120 minutes and the frequency would be between 1 to 3 treatments a day; except in diving accidents where the treatment could be longer. The primary mechanism of action is hyperoxygenation. When the patient breathing oxygen is sustained at 2.0 atm abs, the partial pressure of oxygen in plasma will be close to 1,500 millimeters of mercury (mmHg); and at 3.0 atm abs, it would be are around 2,000 mmHg. In the first minutes of the treatment it causes a small oxidative stress but after the first hour of treatment, there is a supranormal production of enzymatic and non-enzymatic antioxidants; that prevents oxidative stress and damage. This happens when the patient is antioxidant competent. Special attention should be placed on patients that have a condition that could compromise their antioxidant defenses; like chronic renal insufficiency (3,29).
This state of hyperoxygenation produces several secondary mechanisms of action. The increase of oxygen partial pressure generates an increase of oxygen tension. It restores the oxygen to normal or supranormal levels, in the ischemic/hypoxic marginal tissue (penumbra). This effect enhances tissue survival and brakes the edema-hypoxia-edema vicious cycle seen in ischemic/hypoxic tissue. HBOT also generates a vasoconstriction effect. The reduction of around the 30% of the flow will not compromise the tissue oxygenation due to the increased partial pressure of oxygen obtained during the treatment. Vasoconstriction helps to reduce tissue edema and improve microcirculation (3,29). HBOT reduces endothelial damage and restores cell-to-cell junctions (30). It has proven to modulate aquaporins, membrane proteins that form pores and modulate water transport between cells; that dysfunction during ischemia/hypoxia (31).
Through restoration of tissue oxygen tension, HBOT promotes all the active mechanisms that require ATP. Between the most important are the wound healing cascades, angiogenesis, lymphogenesis, and increases growth factor production (3,29). HBOT has direct and indirect antimicrobial effects (29). It promotes bone remodeling (29) and has rheological effects that are synergistic with pentoxifylline (29). It also reduces hypercoagulation induced by zymosan and in MOF (29). Finally, HBOT increases the mobilization of stem cells from bone marrow (3,29).
By restoring oxygen tension and cellular energy production, HBOT restores cellular ion homeostasis, reduces acidosis, stabilizes cellular calcium, and restores mitochondrial oxidative phosphorylation. It also limits excitatory mediators, ROS toxicity, apoptosis, and IRI. If applied in a timely fashion, it salvages the ischemic and metabolic penumbrae (Figure 1) (3,29).
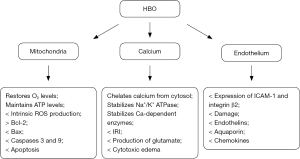
The restoration of tissue, cellular and mitochondrial oxygen tension restores cellular functions. HBOT reestablishes the flux of protons (H+) at the mitochondrial complexes, restoring the production of ATP, reducing the oxidative stress and preventing apoptosis (intrinsic pathway). It limits mitochondrial DNA (mtDNA) damage, caspases expression, and modulates transcription factors (NF-κB, Nogo-A, Ng-R, and RhoA).
HBOT reduces the expression of phospholipase A2, release of calcium, production of COX-2 miRNA, cytokines (IL-1, IL-6, IL-8, and TNF-α), iNOS, and nNOS. Paradoxically, it increases the level of IL-10, an anti-inflammatory cytokine, also mediated by NF-κB. It also promotes hemeoxygenase-1 (HO-1), and heat shock proteins (HSP-70 and 72) (3,29,32,33).
HBOT reduces the expression of adhesion molecules (ICAM-1, VCAM, and integrin β2) and endothelin-1. This modulates its participation in the late phase of IRI, preventing or reducing tissue and organ damage (3,29). In addition to above caused by restoration of oxygenation, it also provides antioxidant protection by inducing selective gene and chaperone production (3). The antioxidant effects are also provided by the increased enzymatic (superoxide dismutase, glutathione peroxidase, Nrf2 and catalase) and non-enzymatic (glutathione and cysteine) antioxidant protection (Figure 2) (3,29).
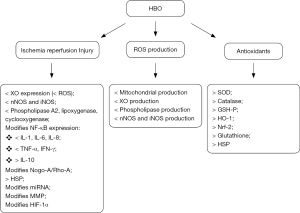
Clinical recommendation for the use of HBOT
HBOT is recommended in the treatment of open fractures and/or with crush injury. Gustilo 3B and 3C injuries are indications for HBOT, but less severe injuries should be considered when there are other risk factors or co-morbidities. HBOT should be starts as early as possible (type I recommendation, level B evidence). The use of HBOT in injuries without fractures is recommended when tissue viability is at risk and there is a high possibility of infection (type 2 recommendation, level C evidence). It is advisable to use HBOT in closed crush injuries and tissue viability is questionable (type 3 recommendation, level C evidence). The use of HBOT in compartment syndrome when the need for fasciotomy is not yet clearly established is a type 3 recommendation, level C evidence. The use of transcutaneous oximetry measurement (TCOM) under pressure may help to determine when the use of HBOT might be recommended (type I recommendation, level B evidence) (34).
A Cochrane review of HBOT and trauma (229 patients) reported a higher complete graft survival associated with HBOT [risk ratio (RR) 3.5; 95% CI: 1.11–2.61] and significantly less tissue necrosis (RR 0.13; 95% CI: 0.02–0.90). Their final recommendation was that further evaluation by means of high quality randomized controlled trials (RCTs) is needed. In a systematic evidence-based review (150 patients) showed beneficial effect of HBOT in crush injuries, with only one complication. The conclusion was that HBOT was not harmful and could be of most benefit if administered early (35,36).
HBOT has been used for acute hypoxic/ischemic injuries for more than 40 years; but despite all the references and meta-analysis published, the medical community is yet hesitant to accepted HBOT as an adjunct treatment option for IRI (3,29).
Hyperbaric oxygen contraindications and side effects
Side effects are very low in patients treated with HBOT. Nevertheless, special care should be taken to avoid them. The most frequent, is middle ear barotrauma and is related to the experience of the personnel operating the chamber. The second in frequency is confinement anxiety. Oxygen toxicity could affect all the body, but it is usually related to the depth (treatment pressure) and the duration of treatment. The most representative oxygen toxicity, is at the CNS. The incidence of CNS oxygen toxicity in HBOT is 1 in 197 treatment at 2.8 atm abs, 1 in 669 treatments at 2.4 atm abs, and 0 in 16,430 treatments at 2.0 atm abs (P<0.001). Lung oxygen toxicity is very rare and could only been observed in long deep treatments for decompression illness (DCI) and in premature neonates. Pediatric patients can be treated the same as adults. The neonatal patients require special care and trained personal to be treated inside a hyperbaric chamber. One of the greatest fears in the treatment of neonates with HBOT is oxygen toxicity. Term neonates have a good anti-oxidant defense; but premature babies with low weight do not; and may be at risk due to their immature antioxidant defense system (3,29,37,38).
The only absolute contraindication for HBOT is untreated tension pneumothorax. Especial care should be taken with patients taking anti-neoplastic medication and with patients with a cardiac dysfunction with a left ventricle ejection fraction below 40. In the first case, cancer medication enhances the production of ROS that could produce oxidative damage when used in conjunction with HBO. In the latter, a low cardiac function could produce pulmonary edema due to an increase in peripheral resistance. Other relative contraindications would be patients with active tumors, although it appears there is no enhancement of tumor growth or angiogenesis with HBOT; and pregnancy, no fetal abnormalities have been reported (3,29).
Conclusions
Hyperbaric oxygen is an accepted treatment for several conditions, including IRI. Any advancement in salvage tissue will make a great difference in the reduction of tissue lost, amputations, quality of life, and global burden of disease; of our patients, their families and society. There is no single treatment that could resolve IRI; its management requires an interdisciplinary group in which HBOT when applied promptly has shown beneficial effects on IRI, including in the CNS (3,29,37).
The adequate and prompt restoration of blood flow in acute IRI will resolve the ischemic penumbra; but it does not impact importantly on the metabolic penumbra. Treatments options that may resolve both, are needed. HBOT has proven to be beneficial as an adjunct treatment in IRI. In order to place HBOT as an accepted adjunct treatment, studies with HBOT applied within 6 h need to produce evidence following the accepted clinical guidelines of the “gold standard treatment”. This could only be accomplished when hyperbaric units are placed inside the trauma centers, and maintain the same quality care of the intensive care units (ICUs) inside the chamber.
HBOT when applied promptly (<6 h) is a safe and effective treatment for IRI. It promotes survival of the metabolic penumbra, restores cellular oxygen levels and function, reduces mitochondrial damage and dysfunction, reduces cytotoxic and tissue edema, and promotes microcirculation. It enhances the healing and regeneration cascades, promotes the production of growth factor, and angiogenesis. It reduces oxidative damage, intrinsic apoptosis cascades, and reduces the production of iNOS. All of these effects are accomplished by restoration of the oxygen tension, cellular oxygen levels, and redox state. As important as these, is the enzymatic and non-enzymatic antioxidant effect that it produces. This is a second line of defenses for the IRI, regardless of the oxygenation accomplish with HBOT. There is a fine line between the benefits, oxidative stress and antioxidant effects of HBOT; it could be referred as the HBOT oxygen balance. Then, it not only saves lives, and tissues; it also reduces morbidity and disability. It modifies favorably the direct and indirect costs, impacting in the global burden of disease caused by IRI.
There is a need for multicenter, multinational, prospective, randomized, and controlled studies, where HBOT is applied in conjunction with the rest of the “gold standard” treatment; within the treatment windows to show its beneficial effects in IRI. HBOT could dramatically change morbidity and mortality and the global burden of the disease and become a very cost-effective adjunct treatment for IRI.
Acknowledgements
I would like to thank my team at Hospital O’Horán (Fernando, Gerardo, Julio, Judith, and Vora) for their unconditional support and for allowing me to have some “free time” to write this manuscript.
Footnote
Conflicts of Interest: The author has no conflicts of interest to declare.
References
- Valparaiso AP, Vicente DA, Bodgrad BA, et al. Modeling acute traumatic injury. J Surg Res 2015;194:220-32. [Crossref] [PubMed]
- Tasoulis MK, Douzinas EE. Hypoxemic reperfusion of ischemic states: an alternative approach for the attenuation of oxidative stress mediated reperfusion injury. J Biomed Sci 2016;23:7. [Crossref] [PubMed]
- Sánchez EC. Mechanisms of action of hyperbaric oxygenation in stroke. Crit Care Nurs Q 2013;36:290-8. [Crossref] [PubMed]
- Penttila A, Trump BF. Studies on the modification of the cellular response to injury III. Electron microscopic studies on the protective effects of acidosis on p-chloromercuribenzene sulfonic acid-(PCMNS) induced injury in Ehrlich ascites tumor cells. Virchows Arch B Cell Pathol 1975;18:17-34. [PubMed]
- Carbonell T. Iron, oxidative stress and early neurological deterioration in ischemic stroke. Curr Med Chem 2007;14:857-74. [Crossref] [PubMed]
- Davidson SM, Yellon DM, Murphy MP, et al. Slow calcium waves and redox changes precede mitochondrial permeability transition pore opening in the intact heart during hypoxia and reoxygenation. Cardiovasc Res 2012;93:445-53. [Crossref] [PubMed]
- Liu F, Lu J, Manaenko A, et al. Mitochondrial in ischemic stroke: new insight and implication. Aging Dis 2018;9:924-37. [Crossref] [PubMed]
- Murphy MP. How mitochondria produce reactive oxygen species. Biochem J 2009;417:1-13. [Crossref] [PubMed]
- Vannucci RC, Duffy TE. Cerebral metabolism in new-born dogs during reversible asphyxia. Ann Neurol 1977;1:528-34. [Crossref] [PubMed]
- Brassai A, Suvanjeiev RG, Bán EG, et al. Role of synaptic and nonsynaptic glutamate receptors in ischemia induced neurotoxicity. Brain Res Bull 2015;112:1-6. [Crossref] [PubMed]
- Balderas E, Zhang J, Stefani E, et al. Mitochondrial BKCa channel. Front Physiol 2015;6:104. [Crossref] [PubMed]
- Elustondo PA, Nichols M, Negoda A, et al. Mitochondrial permeability transition pore induction is linked to formation of the complex of ATPase C-subunit, polyhydroxybutyrate and inorganic polyphosphate. Cell Death Discov 2016;2:16070. [Crossref] [PubMed]
- Sánchez EC. Hyperbaric oxygenation in peripheral nerve repair and regeneration. Neurol Res 2007;29:184-98. [Crossref] [PubMed]
- Sakuma S, Kitamura T, Chihiro K, et al. All-trans arachidonic acid generates reactive oxygen species via xanthine dehydrogenase/ oxidase interconversion in the rat liver cytosol. J Clin Biochem Nutr 2012;51:55-60. [Crossref] [PubMed]
- Leslie CC. Cytosolic phospholipase A2: physiological function and role in disease. J Lipid Res 2015;56:1386-402. [Crossref] [PubMed]
- Milovanovic M, Volarevic V, Radosavljevic G, et al. IL-33/ST2 axis in inflammation and immunopathology. Immunol Res 2012;52:89-99. [Crossref] [PubMed]
- Liu X, Zhang L, Qin H, et al. Inhibition of TRAF3 expression alleviates cardiac ischemia reperfusion injury: a mechanism involving apoptosis, inflammation and oxidative stress. Biochem Biophys Res Commun 2018;506:298-305. [Crossref] [PubMed]
- Marsland AL, Walsh C, Lockwood K, et al. The effects of acute psychological stress on circulating and stimulated inflammatory markers: A systematic review and meta-analysis. Brain Behav Immun 2017;64:208-19. [Crossref] [PubMed]
- Gorsuch WB, Chrysanthou E, Schwaeble WJ, et al. The complement system in ischemia-reperfusion injuries. Immunobiology 2012;217:1026-33. [Crossref] [PubMed]
- Kunz AB, Kraus J, Young P, et al. Biomarkers of inflammation and endothelial dysfunction in stroke with and without sleep apnea. Cerebrovasc Dis 2012;33:453-60. [Crossref] [PubMed]
- Supanc V, Biloglav Z, Kes VB, et al. Role of cell adhesion molecules in acute ischemic stroke. Ann Saudi Med 2011;31:365-70. [Crossref] [PubMed]
- Gillani S, Cao J, Suzuki T, et al. The effect of ischemia reperfusion injury on skeletal muscle. Injury 2012;43:670-5. [Crossref] [PubMed]
- Chang WJ, Toledo-Pereyra LH. Toll-Like receptors signaling in liver ischemia and reperfusion. J Invest Surg 2012;25:271-7. [Crossref] [PubMed]
- McGhan LJ, Jaroszewski DE. The role of toll-like receptor-4 in the development of multi-organ failure following traumatic hemorrhagic shock and resuscitation. Injury 2012;43:129-36. [Crossref] [PubMed]
- Chen Y, Wang L, Zhang L, et al. Inhibition of Connexin 43 hemi channels alleviates cerebral ischemia/reperfusion injury via the TLR4 signaling pathway. Front Cell Neurosci 2018;12:372. [Crossref] [PubMed]
- Yu ZX, Lasaro MA, Bouchard K, et al. Targeting complement pathways during cold ischemia and reperfusion prevents delayed graft function Am J Transplant 2016;16:2589-97. [Crossref] [PubMed]
- Nogueira RC, Bor-Seng-Shu E, Saeed NP, et al. Meta-analysis of vascular imaging features to predict outcome following intravenous rtPA for acute ischemic stroke. Front Neurol 2016;7:77. [Crossref] [PubMed]
- Hu Z, Zhong B, Tan J, et al. The emerging role of epigenetics in cerebral ischemia. Mol Neurobiol 2017;54:1887-905. [Crossref] [PubMed]
- Strauss MB. Crush Injuries and Skeletal Muscle-Compartment. In: Gessell LB. Hyperbaric Oxygen Therapy Indications. 12th edition. The Hyperbaric Oxygen Therapy Committee Report. NC: Undersea and Hyperbaric Medical Society, 2008:39-50.
- Avtan SM, Kaya M, Orhan N, et al. The effects of hyperbaric oxygen therapy on blood-brain barrier permeability in septic rats. Brain Res 2011;1412:63-72. [Crossref] [PubMed]
- Fukuda AM, Badaut J. Aquaporin 4: a player in cerebral edema and neuro inflammation. J Neuroinflammation 2012;9:279. [Crossref] [PubMed]
- Sun L, Strelow H, Miles G, et al. Oxygen therapy improves energy metabolism in focal cerebral ischemia. Brain Res 2011;1415:103-8. [Crossref] [PubMed]
- Novak S, Drenjancevic I, Vukovic R, et al. Anti-Inflammatory Effects of Hyperbaric Oxygenation during DSS-Induced Colitis in BALB/c Mice Include Changes in Gene Expression of HIF-1α, Proinflammatory Cytokines, and Antioxidative Enzymes. Mediators Inflamm 2016;2016:7141430. [Crossref] [PubMed]
- Mathieu D, Marroni A, Kot J. Tenth European Consensus Conference on Hyperbaric Medicine: recommendations for the accepted and non-accepted clinical indications and practice of hyperbaric oxygen therapy. Diving Hyperb Med 2017;47:24-32. [Crossref] [PubMed]
- Eskes A, Vermeulen H, Lucas C, et al. Hyperbaric oxygen therapy for treating acute surgical and traumatic wounds. Cochrane Database Syst Rev 2013.CD008059. [PubMed]
- Garcia-Covarrubias L, McSwain N, VanMeter K, et al. Adjuvant Hyperbaric oxygen therapy in the management of crush injury and traumatic ischemia: an evidence-based approach. Am Surg 2005;71:144-51. [PubMed]
- Sánchez EC. Use of hyperbaric oxygenation in neonatal patients: a pilot study of 8 patients. Crit Care Nurs Q 2013;36:280-9. [Crossref] [PubMed]
- Sánchez EC. Management of Acute Ischemic Hypoxic Encephalopathy in Newborns with Hyperbaric Oxygen: A Review. Austin J Cerebrovasc Dis & Stroke 2016;3:1049.
Cite this article as: Sánchez EC. Pathophysiology of ischemia-reperfusion injury and its management with hyperbaric oxygen (HBO): a review. J Emerg Crit Care Med 2019;3:22.